Overview
Our research is focused on the emergence and nature of collective phenomena in quantum many-body systems. Ultracold atomic gases offer powerful platforms for emulating many-body behaviour in regimes which are difficult to access theoretically, such as the strongly-correlated physics of superconductors and quantum Hall fluids, and the properties of quantum matter driven away from equilibrium. Using ultracold atomic gases combined with the exquisite control over potentials and interactions afforded by the AMO toolbox, we build both toy models of paradigmatic many-body systems found in nature, as well as realise entirely new states of matter.
Er-Li: We are currently building our first apparatus, designed to produce a quantum degenerate mixture of erbium and lithium atoms. This species choice confers exciting possibilities for realising novel quantum phases: lithium is the paradigmatic element for addressing strongly-correlated Fermi gases, with convenient Feshbach resonances for tuning interactions and excellent collisional stability. Erbium features strong long-range magnetic interactions, large spin, and its atomic level structure makes it well suited for the generation of laser-induced gauge fields. Together with the extreme mass imbalance and interspecies Feshbach resonances, this combination offers numerous directions including exploiting phonon-exchange for new forms of long-range interactions, mixed dimensional systems, collective spin physics, topological quantum matter, tests of few body Efimov physics, and new classes of ultracold molecules. The quantum gas is prepared inside a nano-textured glass cell, and is imaged and manipulated using a high-resolution microscope objective.
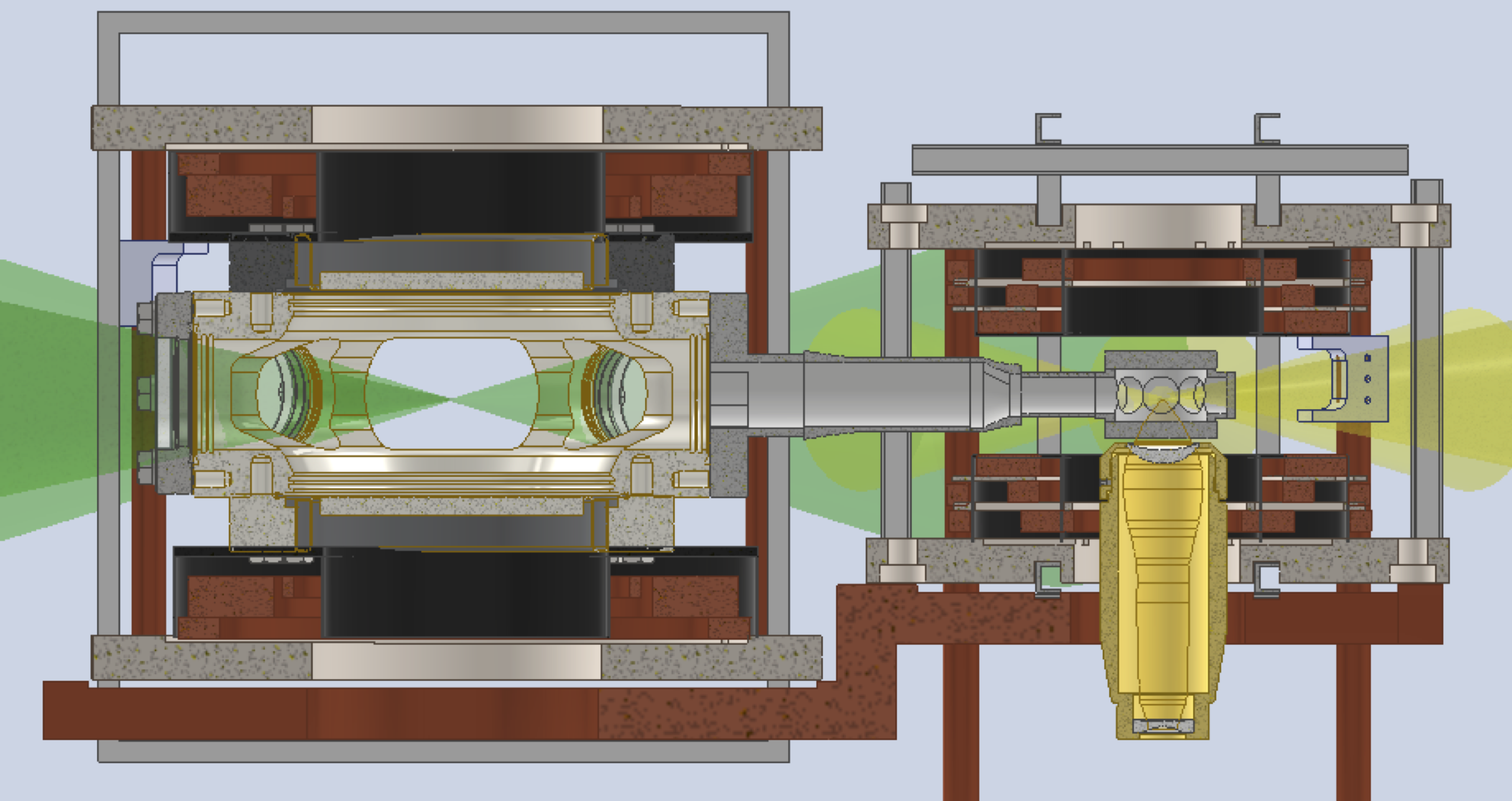
Previous projects
Spontaneous crystallization of a quantum Hall state
Self- organization of matter is ubiquitous throughout nature, from structure formation in living systems, emergence of weather patterns, galaxy formation, and the magneto-hydrodynamic instabilities of charged plasmas. Of crucial importance is to uncover the general rules governing how pattern formation arises from the competition between different energy and lengthscales, and the dynamical instabilities which generically seed transitions to a broken-symmetry state.
In correlated quantum matter such as quantum Hall liquids, atoms in optical lattices, and twisted bilayer graphene, interactions and fluctuations often compete to drive phase transitions. A prime example occurs for electrons in strong magnetic fields. The single particle states form discrete, highly degenerate Landau levels, and correspond to wavepackets localized to the magnetic length. In the presence of interactions between electrons, owing to the absence of kinetic energy, one naturally expects the formation of a Wigner crystal. Famously however, the interplay of the macroscopic degeneracy and interactions instead typically favors the strongly correlated quantum Hall liquids, which host fractional charges, anyonic exchange statistics, and topologically protected transport properties. The tendency to crystallize is still apparent in a pronounced minimum in the collective excitation spectrum. In analogy with the roton minimum in 4He, also considered a precursor of solidification, these excitations are called magneto-rotons.
In this work with the Zwierlein group, published in Nature, we applied a strong artificial magnetic field to a Bose-Einstein condensate via rapid rotation of its reference frame. We coaxed the condensate to occupy a single Landau gauge wavefunction in the lowest Landau level, a textbook quantum mechanical object, and investigated its purely interaction-driven behavior. We revealed a spontaneous crystallization of the fluid, driven by the proliferation of magneto-rotons, and confirmed the singular role of interactions in determining the rate of dynamics, which is a smoking gun of lowest Landau level physics. Remarkably, by simply increasing the superfluid density, this quantum mechanical instability connects smoothly to a classical Kelvin-Helmholtz type fragmentation also displayed by line plumes in atmospheric and oceanic systems, hadron beams in cyclotrons, and charged plasmas, connecting pattern formation across 9 orders of magnitude in lengthscale.
Squeezing of spatial Heisenberg uncertainty in a synthetic magnetic field
In the quantum description of particles in a magnetic field, translations along orthogonal spatial directions do not commute. This underlies the Hall effect, determines the zero-point extent to cyclotron orbits, mediates the incompressibility of fluids moving in a magnetic field, and implies a Heisenberg uncertainty relation between spatial coordinates. Working with the Zwierlein group, we performed quantum mechanical squeezing of this geometric Heisenberg uncertainty using a Bose-Einstein condensate under rotation, in which the Coriolis force plays the role of the Lorentz force. This squeezing induces a dynamical transformation into a condensate occupying a single Landau gauge wavefunction in the lowest Landau level. Using high-resolution microscopy we directly resolved the zero-point cyclotron orbits, and demonstrated squeezing of space by more than 7 decibels below the standard quantum limit.
This work, published in Science, opened up a new route to strongly-correlated bosonic quantum Hall physics. Using a Landau gauge rather than symmetric gauge starting point obviates delicate fine-tuning of the trap isotropy, which has presented the main challenge to previous quantum gas approaches. We can now straightforwardly produce gases with an interparticle spacing comparable to the cyclotron orbit size, signaling the onset of strongly-correlated physics. The engineering of bosonic quantum Hall states heralds an entirely new field of many-body physics, having no counterpart in nature.